Manufacturing of Electrocatalytic Material for Large Scale Hydrogen Production by the Combination of Electroplating, PVD, Thermochemical Diffusion and Leaching Processes
This paper is a fine example of the multitude of ways that surface finishing can be brought to bear in process development. In this case, a combination of processes is applied to large scale hydrogen production.
by Cecilía Kjartansdóttir,a Michael Caspersen,b Sune Egelund,b Lars Pleth Nielsenc and Per Møllera
aTechnical University of Denmark, Lyngby, Denmark
bSiemens A/S Denmark
cDanish Technological Institute, Aarhus, Denmark
Editor’s Note: This paper is a peer-reviewed and edited version of a presentation delivered at NASF SUR/FIN 2014 in Cleveland, Ohio on June 10, 2014. A printable PDF version is available by clicking HERE.
ABSTRACT
Novel high performance electrodes for industrial alkaline water electrolysis have been manufactured by the physical vapor deposition of aluminum onto a nickel-plated substrate. Nanoporous high surface area nickel is obtained by thermochemical diffusion followed by selective leaching of aluminum. The developed porous nickel surface is uniform and has good interlayer adhesion due to its chemical bonding properties. Electrochemical measurements reveal that 400 mV less potential is needed to decompose water into hydrogen and oxygen with the developed electrodes as compared to a solid nickel electrode. High resolution scanning electron microscope (HR‐SEM) photographs reveal nickel-electrode surfaces characterized by a large surface area with pores down to a few nanometers in size. Durability testing (>9,000 hr) was carried out in a commercially produced electrolyzer stack, revealing no serious deactivation or decomposition during the test period.
Keywords: Electrode production, electrolysis of water, hydrogen production, multi-step surface finishing, electroplating, physical vapor deposition, thermochemical diffusion, leaching
Introduction
Energy demand worldwide will, according to the World Energy Outlook,1 increase by one-third from 2011 to 2035. Accordingly, in order to meet this ever increasing energy demand and diminish the risk of dramatic climate change in the coming years, a rapid shift in the global energy trend away from coal and fossil fuel is necessary.
Renewable energy is commonly recognized to be the answer for a more secure, reliable and sustainable future. Combining the production of hydrogen via water electrolysis with renewable energy sources offers the possibility of increased production capacity and load management with no greenhouse emissions. Hydrogen as an energy carrier comprises the advantage of flexibility, being able to contribute to various kinds of energy sources. The most obvious route is the production of hydrogen with excessive power from fluctuating renewables such as wind turbines and solar cells followed by storage and later production of electricity via fuel cells or gas turbines, when needed. The hydrogen can also be used as a raw material for production of synthetic fuels, such as methane by the Sabatier process2 and liquid fuels by the Fischer-Tropsch synthesis.3
Among the commercially available water electrolysis systems, alkaline water electrolysis (AWE) comprises by far the highest production rate and the lowest production cost, making the technique the current standard for large‐scale water electrolysis systems.4,5 Commercial alkaline electrolyzers are typically operated in a liquid electrolyte containing 25‐40% potassium hydroxide at a temperature ranging from 60 to 90°C.6,7,8
The highly alkaline electrolyte, the intermediate temperature and the oxygen and hydrogen gas evolution all contribute in making the electrode media extremely corrosive. Thus, finding low cost electrode materials that are both efficient and have long‐term stability is one of the remaining challenges within the field of AWE.
There are two ways to increase the efficiency of an electrode: (1) increasing the electrocatalytic properties of the electrode surface, by selecting materials that are good catalysts for the reactions involved, or (2) increasing the specific surface area of the electrode. Both of these result in a reduction of the necessary voltage to draw the current applied.
Among non‐noble metals, nickel is one of the most stable metals in strong alkaline solutions. Nickel also has relatively good electrocatalytic properties for hydrogen and oxygen formation. Nickel or nickel plated substrates are therefore typically the core material used in electrodes for AWE systems.5,8,9 The electrochemical efficiency of the nickel electrodes can be improved even further by increasing the number of active sites, by increasing the actual surface area and by introducing additional surface defects.10-18
A common method for preparing high surface area nickel catalysts is by selectively alkaline leaching aluminum or zinc from a Ni‐Al or a Ni‐Zn alloy, respectively. This method of increasing the surface area of nickel by alkaline leaching was first introduced by Murray Raney in 1925 for the purpose of producing a catalyst for the hydrogenation of vegetable oils.19 However, the production of a durable electrocatalyst for AWE is much more challenging than for non‐electrochemical processes such as hydrogenation. The reason for this is the risk of gas‐erosion of the electrocatalytic surfaces during AWE because of the hydrogen and oxygen evolution on the electrodes. Hence, when choosing the right process technique for electrode development, the interlayer adhesion between the porous structure and the substrate, as well as the purity, i.e., elimination of oxides, of the final product must be taken into consideration.
In the present work, a state‐of‐the‐art electrode has been produced by the physical vapor deposition of aluminum onto an electroplated nickel substrate, followed by thermochemical diffusion and selective alkaline leaching. The efficiencies of the electrodes were investigated by steady state polarization measurements and measurements in a commercial electrolysis stack.
Experimental
Preparation of electrodes
Electrodeposited sulfamate nickel coatings are known to have low stress and high ductility. These properties could benefit the durability of the nickel electrodes. Thus, here the sulfamate nickel coating was selected over other nickel coatings. The sulfamate nickel coating was electroplated onto a 50×50 mm2 304 stainless steel substrate in an electrolyte containing 300 g/L Ni(SO3NH2), 40 g/L H3BO3 and 10 g/L NiCl2•6H2O. The deposition current density was 4.5 A/dm2 and the plating duration was 2 hr at 45°C. The pretreatment of the steel substrate was as follows: cathodic degreaser for 2 min, pickling for 1 min, etching in 20% HCl and 5% H2O2 for 1 min (to remove chromium oxides from the surface), followed by cathodic degreasing for 2 min and pickling for 1 min. In order to assure good adhesion, a thin layer of Woods nickel strike was electroplated onto the steel substrate according to the following sequence: anodic at 7 A/dm2 for 2 min (to remove the last oxides on the surface) and cathodic deposition at 14 A/dm2 for 2 min.
The nickel plated steel substrates were coated with approximately 20 μm of aluminum by DC magnetron sputtering using a CC800/9 SinOx coating unit from CemeCon AG. The aluminum source consisted of four aluminum 1050 targets, run at 750 W. The bias on the substrate was set to 800 W and the start pressure in the chamber was 1 mPa. The nickel surfaces were cathodically degreased for 2 min prior to the PVD process. The substrates were heated and etched in situ by argon sputtering, prior to the sputtering process, to remove any nickel oxide (NiO) from the surface. The aluminum PVD specimens were subsequently heat treated in an atmospheric furnace for 4 hr at 610°C, followed by selective aluminum leaching in 30 wt% KOH and 10 wt% KNaC4H4O6•4H2O at 80°C with stirring for 24 hr. The reason for adding the sodium potassium tartrate tetrahydrate to the alkaline leaching solution is that it works as a complexing agent for the leached aluminum according to:20
Al(OH)4- + n(C4H4O6)-2 ⇒ Al(OH)3(C4H4O6)n-2 + OH-,
thereby preventing the aluminum hydroxide precipitates from redepositing into the nickel pores:21
Al(OH)4- ⇒ Al(OH)3 + OH-
Structural characterization and composition
For cross-section characterization and composition analyses the coated specimens were hot‐mounted in PolyFast from Struers, ground down to 4000 grit and subsequently polished with 3 and then 1 μm diamonds. The cross-section studies were made with a TM 3000 tabletop scanning electron microscope (SEM) from Hitachi. An integrated energy‐dispersive x‐ray spectroscopy (EDS) was used for elemental analyses. The surface structure and morphology of the electrode coating was prepared in the same manner as described before, except that the substrate was made of solid nickel, and was characterized by means of a FEI Quanta 200 ESEM FEG‐SEM.
Electrochemical and durability measurements
The electrocatalytic activity of the electrodes was evaluated by means of anodic and cathodic potentiodynamic polarization. The measurements were carried out using a Gamry Reference 3000 potentiostat. A typical three-electrode electrochemical cell made of Teflon was used for the electrochemical measurements. The cell contained a Hg/HgO reference electrode from Radiometer Analytical and a counter electrode made of pure nickel. The electrolyte consisted of 1M KOH and the measurements were carried out at 25°C. The potentiodynamic and cyclic voltammetry measurements were IR‐compensated by means of a current interruption technique. All following half‐cell potentials are reported vs. Hg/HgO. A durability test was carried out in a 17-cell bipolar, non‐zero gap alkaline water electrolysis stack manufactured by GreenHydrogen.dk. The electrodes in the stack measured 270 cm2 in area. The stack contained 30% KOH and was operated under altered conditions, at a maximum temperature of 80°C and a pressure of 22 bar for more than 9,000 hr. As the electrodes were treated with the alkaline-leach etched PVD Al/Ni on both sides (Here the nickel substrate was of solid nickel plates.), the developed surface functioned both as anode and cathode in the electrolysis stack.
Results and discussion
Structural characterization and composition
When heat‐treated at 610°C, a thermochemical diffusion process takes place at the interface between the aluminum and the nickel. The red horizontal line in the phase diagram of Fig. 1 indicates which Ni‐Al diffusion couples are thermodynamically stable at 610°C and atmospheric pressure. The thickness of each intermetallic phase formed depends on the diffusion kinetics, the amount of nickel and aluminum available in the diffusion system and the heat treatment parameters.
Among Al/Ni alloys, aluminum can only be alkaline leached from the Al3Ni2 and Al3Ni phases.23 Obviously, the Al3Ni2 phase contains more nickel and is therefore more mechanically stable compared to its Al3Ni counterpart. Hence, the aim during the alloy formation procedure, i.e., the thermochemical diffusion process, was to obtain a relatively thick layer of the Al3Ni2 phase.
Figures 2 and 3 show SEM micrographs of the PVD aluminum nickel structure after 4 hr of heat treatment and after heat treatment followed by selective aluminum leaching. The results from the EDS analyses performed on the cross‐sections are listed in Table 1.

Figure 1 - Phase diagram for the Al-Ni diffusion couples adapted from Ref. 22. The horizontal line indicates the thermochemical diffusion temperature (610°C) selected for the for the electrode coating.

Figure 2 - Cross section SEM micrographs of PVD aluminum on a sulfamate nickel substrate heat treated at 610°C for 4 hr.

Figure 3 - Cross section SEM micrographs of PVD aluminum on a sulfamate nickel substrate heat treated at 610°C for 4 hr and alkaline aluminum leached.
Table 1 - EDS analyses on the PVD aluminum on sulfamate nickel specimens (Figs. 2 and 3).
It is evident from the SEM micrographs and the EDS analysis that the majority of the aluminum in the Al3Ni2 phase is leached during the leaching procedure. Hence, the aluminum content decreases from ~39 wt% to ~7 wt%. The high oxygen content in the porous layer is most likely due to resin trapped inside the pores. No cracks are visible in the porous structure.
For nanostructure investigations, high‐resolution SEM studies are required. A high resolution SEM micrograph of the surface of the developed coating is shown in Fig. 4. From the HR‐SEM image it is evident that the coating developed is highly porous with pores down to the nanometer scale.

Figure 4 - HR‐SEM surface micrograph of the developed porous coating.
Electrochemical measurements and durability testing
Results from the potentiodynamic measurements recorded on the developed Al/Ni coating compared to solid nickel electrodes are shown in Figs. 5 and 6. It is evident from the steady‐state polarization measurements that the developed coating has 360 mV less hydrogen overpotential and 50 mV less oxygen overpotential as compared to solid nickel at a current density of 0.6 A/cm2, corresponding to 60 A/dm2. This implies that less voltage is needed to draw the current applied for formation of hydrogen in an alkaline electrolyte consisting of 1M KOH at 25°C. That means that each cell can produce hydrogen with a reduction of 410 mV in cell voltage as compared to pure nickel. This again implies that the total cell voltage with a pure nickel electrode shows a voltage of 2.35 V, compared with the developed electrodes, which only shows 1.94 V. In practice that saves about 17% of the energy for the formation of hydrogen, which is of considerable importance for the economy, particularly when the energy is produced by wind turbines and/or solar cells.

Figure 5 - Cathodic polarization curves recorded on the developed coating compared to solid nickel measured in a 1M KOH electrolyte at 25°C.

Figure 6 - Anodic polarization curves recorded on the developed coating compared to solid nickel measured in a 1M KOH electrolyte at 25°C.
A durability test was carried out in a commercially available 17 cell bipolar, non‐zero gap alkaline water electrolysis stack manufactured by GreenHydrogen.dk. Figure 7 shows an exploded CAD drawing of the electrolysis. In Fig. 8, the stacking of the electrodes, bipolar non‐zero gap stacking, inside the electrolyzer is illustrated.
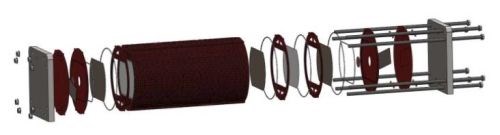
Figure 7 - CAD image of the construction of the electrolysis stack used for durability testing. Courtesy of GreenHydrogen.dk.

Figure 8 - The bipolar configuration of the electrolysis stack used for durability testing. Courtesy of GreenHydrogen.dk.
The durability test was performed in combination with a demonstration project named H2‐College. In the project, 66 houses at the campus of Aarhus University in Herning, Denmark were powered by hydrogen, where surplus power from wind turbines was used to generate the electricity for the electrolysis process. The durability test was carried out for approximately 9,000 hr. No indications of deactivation of the electrodes were observed during the operating period. Images of the electrolysis stack and the electrolysis system are shown in Figs. 9 and 10, respectively. Figure 11 shows the temperature versus average cell voltage measured after 9,000 hr of operation. Further information about H2‐College can be found elsewhere.24,25

Figure 9 - Image of the electrolysis stack produced by GreenHydrogen.dk. Courtesy of GreenHydrogen.dk.

Figure 10 - Image of the electrolysis system (with two electrolysis stacks installed) produced by GreenHydrogen.dk. Courtesy of GreenHydrogen.dk.

Figure 11 - Temperature versus voltage measured on the 17‐cell bipolar electrolysis stack at 22 bar and a current density of 200 mA/cm2, after approximately 9,000 operating hours, showing the temperature dependence of the averages cell voltage. The efficiency calculations are based on the HHV (1.48V).
A cell efficiency of about 81% is observed at 200 mA/cm2, 80°C and 22 bar. The efficiency values from the stack measurements are, however, most likely lower than the actual cell efficiencies due to losses in the stack itself.
Conclusions
In the present study, high surface area nickel electrode coatings have been produced by combining physical vapor deposition of aluminum onto an electroplated nickel substrate followed by thermochemical diffusion and selective aluminum leaching. Electrochemical and durability measurements of the developed coating show that 17% less energy is needed to decompose water into hydrogen and oxygen over the developed porous nickel electrodes. Durability tests have been performed in a commercially available electrolysis stack, for over 9,000 hr, where no serious deactivation of the electrode efficiency was observed.
References
1. OECD World Energy Outlook 2013, International Energy Agency, Paris (2013); http://www.iea.org/Textbase/npsum/WEO2013SUM.pdf (last accessed 12/05/2014).
2. F. Mohseni, et al., Applied Energy, 90 (1), 11-16 (2012).
3. C.A. Floudas, J. A. Elia & R.C. Baliban, Computers & Chem. Eng., 41, 24-51 (2012).
4. K. Harrison & J.I. Levene, “Electrolysis of Water,” in: Solar Hydrogen Generation: Toward a Renewable Energy Future, K. Rajeshwar, R. McConnell & S. Licht, Eds., Springer, New York, 2008; pp. 41-63.
5. Renewable Hydrogen Technologies: Production, Purification, Storage, Applications and Safety, 1st Edition, L. Gandia, G. Arzamedi & P. Dieguez, Eds., Elsevier, Waltham, Mass., 2013.
6. D.A.J. Rand & R.M. Dell, Hydrogen Energy: Challenges and Prospects, Royal Society of Chemistry, London, UK; Washington, DC, 2007.
7. C.K. Dyer, et al., Encyclopedia of Electrochemical Power Sources, Newnes (Elsevier), Oxford, UK; Boston, MA, 2013.
8. K. Zeng & D. Zhang, Prog. Energy & Combustion Sci., 36 (3), 307-326 (2010).
9. T. Smolinka, “Fuels - Hydrogen Production / Water Electrolysis,” in Encyclopedia of Electrochemical Power Sources, J. Garche, Ed., Elsevier, Amsterdam, 2009; pp. 394–413.
10. S.M.A. Sillitto, et al., “Characterisation of Advanced Raney Nickel Electrocatalytic Coatings Produced by the Direct Spraying Method,” Inst. of Chemical Engineers Symposium Series, 145, 101-110, Inst. Chem. Eng., Rugby, UK, 1999.
11. S.M.A. Sillitto, et al., “Electrochemical Testing and Structural Characterisation of Nickel Based Catalytic Coatings Produced by Direct Spraying, “in MRS Proceedings-Fall 1998, Symposium on Advanced Catalytic Materials, Vol. 549, P.W. Lednor, D.A. Nagaki & L.T. Thompson, Eds., Materials Research Society, Warrendale, PA, 1999; pp. 23-29.
12. A. Kellenberger, et al., Int. J. Hydrogen Energy, 32 (15), 3258-3265 (2007).
13. A. Kellenberger, N. Vaszilcsin & W. Brandl, J. Solid State Electrochem., 11 (1), 84-89 (2007).
14. L. Birry & A. Lasia, J. Appl. Electrochem., 34 (7), 735-749 (2004).
15. A. Kellenberger & N. Vaszilcsin, Rev. Chim., 56 (7), 712-715 (2005).
16. G. Schiller, R. Henne & V. Borck, J. Therm. Spray Technol., 4 (2), 185-194 (1995).
17. J. Fournier, D. Miousse & J.G. Legoux, Int. J. Hydrogen Energy, 24 (6), 519-528 (1999).
18. T. Boruciński, S. Rausch & H. Wendt, J. Appl. Electrochem., 22 (11), 1031-1038 (1992).
19. M. Raney, U.S. Patent 1,628,190 (1927).
20. H.L. Watts & D.W. Utley, Anal. Chem., 25 (6), 864-867 (1953).
21. Y. Li, et al., Hydrometallurgy, 98 (1-2), 52-57 (2009).
22. M.F. Singleton, J.L. Murray & P. Nash, “Al‐Ni (Aluminum‐Nickel),” in Binary Alloy Phase Diagrams, Vol. 1, T.B. Massalski, et al., Eds., ASM International, Materials Park, OH, 1986; p. 142.
23. M.L. Bakker, D.J. Young & M.S. Wainwright, J. Mater. Sci., 23 (11), 3921-3926 (1988).
24. “The H2College project,” http://greenhydrogen.dk/Default.aspx?ID=551 (last accessed 12/06/2014).
25. “Climate Change and Innovation in the Building Sector: H2-College in Herning,” http://www.climatebuildings.dk/h2college.php (last accessed 12/06/2014).
About the authors
Related Content
Trivalent Chrome Overview
As the finishing industry begins to move away from the use of hexavalent chromium to trivalent chromium, what factors should finishers consider as they make new investments? Mark Schario, chief technology officer for Columbia Chemical offers a helpful overview of this complicated topic.
Read MoreInnovation in Plating on Plastic
Plating on advanced plastics solution offers improved adhesion, temperature resistance and cost savings.
Read MoreProducts Finishing Reveals 2024 Qualifying Top Shops
PF reveals the qualifying shops in its annual Top Shops Benchmarking Survey — a program designed to offer shops insights into their overall performance in the industry.
Read MoreLiquid Chrome Vs. Chromic Acid Flake
Contemplating how to continue offering chromic acid services in an increasingly stringent regulatory world? Liquid chrome products may be the solution you’re looking for.
Read MoreRead Next
Education Bringing Cleaning to Machining
Debuting new speakers and cleaning technology content during this half-day workshop co-located with IMTS 2024.
Read MoreA ‘Clean’ Agenda Offers Unique Presentations in Chicago
The 2024 Parts Cleaning Conference, co-located with the International Manufacturing Technology Show, includes presentations by several speakers who are new to the conference and topics that have not been covered in past editions of this event.
Read MoreEpisode 45: An Interview with Chandler Mancuso, MacDermid Envio Solutions
Chandler Mancuso, technical director with MacDermid Envio discusses updating your wastewater treatment system and implementing materials recycling solutions to increase efficiencies, control costs and reduce environmental impact.
Read More